Battery Storage
Battery storage is essential to a fully-integrated clean energy grid, smoothing imbalances between supply and demand and accelerating the transition to a carbon-free future.
Many innovators built our understanding of electricity...
On its most basic level, a battery is a device consisting of one or more electrochemical cells that convert stored chemical energy into electrical energy.
Each cell contains a positive terminal, or cathode, and a negative terminal, or anode. Electrolytes allow ions to move between the electrodes and terminals, which allows current to flow out of the battery to perform work.
Advances in technology and materials have greatly increased the reliability, output, and density of modern battery systems, and economies of scale have dramatically reduced the associated cost. Continued innovation has created new technologies like electrochemical capacitors that can be charged and discharged simultaneously and instantly and provide an almost unlimited operational lifespan. The following pages offer greater insight into these technologies and the many applications that they are utilized for in creating a more robust and adaptable energy grid.
Battery storage at a glance
1,756
$27M
$5.1B
Lithium Ion (Li-Ion) batteries
After Exxon chemist Stanley Whittingham developed the concept of lithium-ion batteries in the 1970s, Sony and Asahi Kasei created the first commercial product in 1991.
The first batteries were used for consumer electronics and now, building on the success of these Li-ion batteries, many companies are developing larger-format cells for use in energy-storage applications. Many also expect there to be significant synergies with the emergence of electric vehicles (EVs) powered by Li-ion batteries. The flexibility of Li-ion technology in EV applications, from small high-power batteries for power buffering in hybrids, to medium-power batteries providing both electric-only range and power buffering in plug-in hybrids, to high-energy batteries in electric-only vehicles, has similar value in stationary energy storage.
Redox Flow Batteries (RFB)
Redox flow batteries (RFB) represent one class of electrochemical energy storage devices.
The name “redox” refers to chemical reduction and oxidation reactions employed in the RFB to store energy in liquid electrolyte solutions which flow through a battery of electrochemical cells during charge and discharge.
The total difference in chemical potential between the chemical states of the active elements on the two sides of the battery determines the electromotive force (emf or voltage) generated in each cell of the battery. The voltage developed by the RFB is specific to the chemical species involved in the reactions and the number of cells that are connected in series. The current developed by the battery is determined by the number of atoms or molecules of the active chemical species that are reacted within the cells as a function of time. The power delivered by the RFB is the product of the total current and total voltage developed in the electrochemical cells. The amount of energy stored in the RFB is determined by the total amount of active chemical species available in the volume of electrolyte solution present in the system.
How Redox Flow Batteries Work
The separation of power and energy is a key distinction of RFBs, compared to other electrochemical storage systems. As described above, the system energy is stored in the volume of electrolyte, which can easily and economically be in the range of kilowatt-hours to tens of megawatt-hours, depending on the size of the storage tanks. The power capability of the system is determined by the size of the stack of electrochemical cells. The amount of electrolyte flowing in the electrochemical stack at any moment is rarely more than a few percent of the total amount of electrolyte present (for energy ratings corresponding to discharge at rated power for two to eight hours). Flow can easily be stopped during a fault condition. As a result, system vulnerability to uncontrolled energy release in the case of RFBs is limited by system architecture to a few percent of the total energy stored. This feature is in contrast with packaged, integrated cell storage architectures (lead-acid, NAS, Li Ion), where the full energy of the system is connected at all times and available for discharge.
The separation of power and energy also provides design flexibility in the application of RFBs. The power capability (stack size) can be directly tailored to the associated load or generating asset. The storage capability (size of storage tanks) can be independently tailored to the energy storage need of the specific application. In this way, RFBs can economically provide an optimized storage system for each application. In contrast, the ratio of power to energy is fixed for integrated cells at the time of design and manufacture of the cells. Economies of scale in cell production limit the practical number of different cell designs that are available. Hence, storage applications with integrated cells will usually have an excess of power or energy capability.
RFBs can be divided into two categories: 1) true redox flow batteries, where all of the chemical species active in storing energy are fully dissolved in solution at all times; and 2) hybrid redox flow batteries, where at least one chemical specie is plated as a solid in the electrochemical cells during charge. Examples of true RFBs include the vanadium-vanadium and iron-chromium systems. Examples of hybrid RFBs include zinc-bromine and zinc-chlorine systems.
Complete Separation of Power and Energy
True RFBs achieve the complete separation of power and energy, along with the full advantages.
In hybrid RFBs, complete separation of power and energy is not achieved, because energy is stored in the metal which is plated in the electrochemical stack during charge. Larger energy storage capacity requires a larger stack, so the distinction of the hybrid RFB from integrated cell architectures is only partly achieved.
Finally, RFBs are well suited for applications with power requirements in the range of tens of kilowatts to tens of megawatts, and energy storage requirements in the range of 500 kilowatt-hours to hundreds of megawatt-hours. RFBs can be the most economical choice in this range because storage tanks and flow controls are easy and economical to scale, and electrochemical stacks can have repeat units with power ratings in the tens to hundreds of kilowatts.
Redox flow batteries have one main architectural disadvantage compared with integrated cell architectures of electrochemical storage. RFBs tend to have lower volumetric energy densities than integrated cell architectures, especially in the high power, short duration applications. This is due to the volume of electrolyte flow delivery and control components of the system, which is not used to store energy, so a system is not as compact as other technologies might be for a similar output. In spite of this, RFBs are available with system footprint below the EPRI substation target of <500 ft2 / MWh.
Redox flow batteries offer an economical, low vulnerability means to store electrical energy at grid scale. Redox flow batteries also offer greater flexibility to independently tailor power rating and energy rating for a given application than other electrochemical means for storing electrical energy. Redox flow batteries are suitable for energy storage applications with power ratings from tens of kW to tens of MW and storage durations of two to 10 hours.
Lead batteries
Lead batteries are the most extensively used rechargeable battery technology in the world.
They have an unrivalled track record for reliability and safety, which together with a well-established worldwide supplier base, make them the dominant battery in terms of MWh of production. Lead batteries are widely used in cars and trucks, being used in virtually all vehicles, supporting increased vehicle hybridization and electrification, all the way from start-stop technology to full electric vehicles. In addition, lead batteries are widely used in industrial applications, where they provide energy for telecommunications, uninterrupted power supply, secure power, electric traction and for energy storage for utilities as well as domestic and commercial applications.
Why lead batteries make sense for energy storage
Lead batteries have a long history of successful use in energy storage and their capabilities and limitations have been carefully researched. Their reliability is well established, and they can be adapted for a wide range of duty cycles which will ensure they provide a good solution that is competitive with other approaches.
In addition, lead batteries are widely recycled. Thanks to its long-established collection and recycling scheme, almost all used lead batteries are collected at end-of-life for recycling – the highest of all battery technologies.
Lead batteries exemplify the fundamental principles of eco-design: they are designed to be recycled at end-of-life with more than 90% of their material being recovered. The average lead battery made today contains more than 80% recycled materials, and almost all of the lead recovered in the recycling process is used to make new lead batteries.
For energy storage applications the battery needs to have a long cycle life both in deep cycle and shallow cycle applications. Deep cycle service requires high integrity positive active material with design features to retain the active material. Shallow cycle service places more stress on the negative active material and the battery has to be designed so that sulfation is avoided. Charging and battery management is important and energy efficiencies of 90% can be achieved.
The Consortium for Battery Innovation (CBI) is an industry funded pre-competitive research and market development organization. CBI has been actively supporting new developments for lead batteries for more than 25 years which has played an important part in improving cycle life under different conditions.
Lead batteries may be used for frequency regulation and for load management for utilities and are widely deployed to support PV installations both in commercial and domestic premises. Best in class lead batteries can achieve 5000 cycles to 70% depth-of-discharge which will provide close to 15 years life when used intensively. Lead batteries have lower costs than other chemistries and, at end-of-life, have a positive value for the lead metal available for recycling. They are also safe as they have an aqueous electrolyte and active materials that are not flammable. Li-ion batteries have a flammable organic electrolyte and highly reactive component materials. Safety engineering is important for all battery systems but for Li-ion very high standards are required.
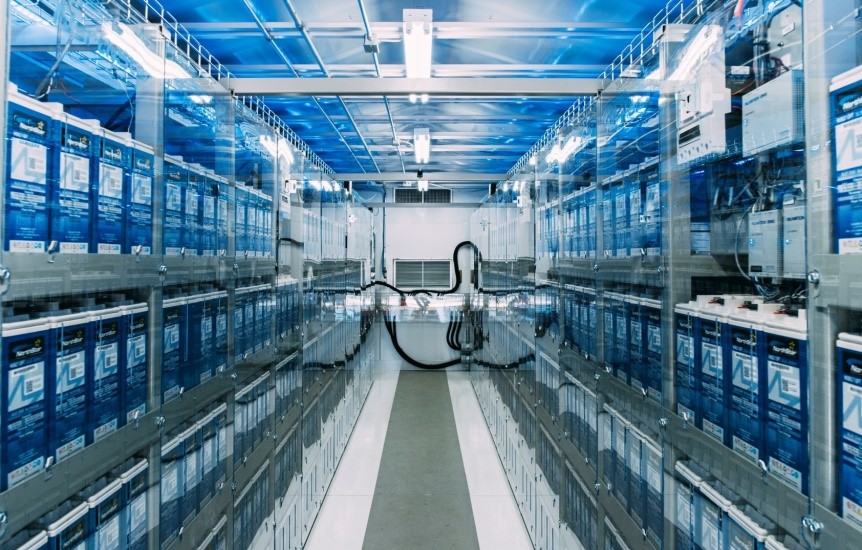
Technical Information
Lead battery chemistry is simple and robust. The active material is lead dioxide on the positive plates, and finely divided lead on the negative plates. Both of these materials react with sulfuric acid on discharge to form lead sulfate and water and the reverse reactions take place on recharge.
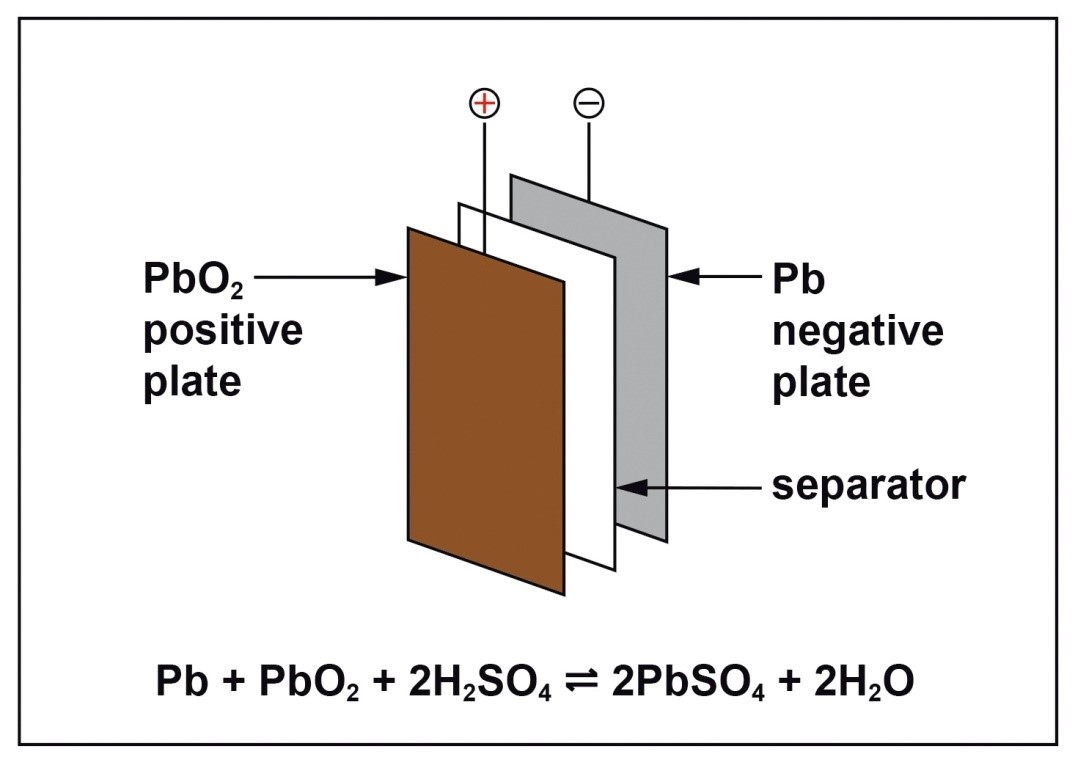
Lead batteries for energy storage are made in a number of different types. They can be flooded which means that they require maintenance additions of water from time to time or valve-regulated lead-acid (VRLA) types which require no routine maintenance other than safety inspections. VRLA batteries are constructed such that hydrogen evolution is suppressed and oxygen is chemically recombined so that water loss is virtually eliminated. There are two technologies available; one uses an absorptive glass mat (AGM) as the separator and the other uses finely divided silica to gel the electrolyte. They both operate in a similar way but have different characteristics. Lead batteries also have different designs for particular applications and are mainly differentiated by the positive plate which may be tubular or a flat pasted plate.
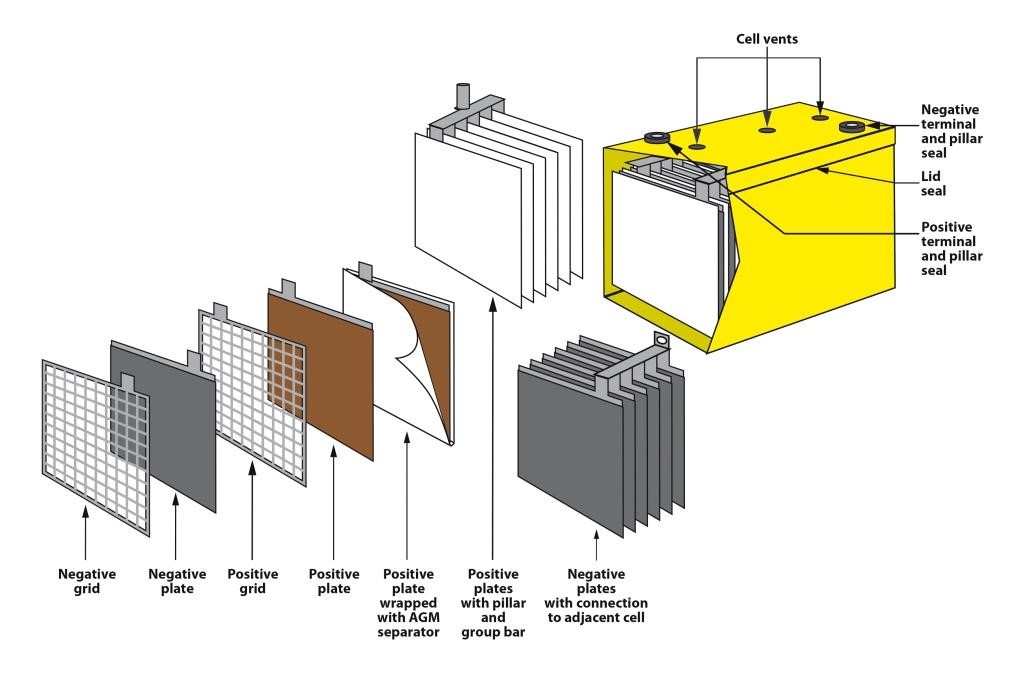
Vanadium Redox (VRB) Flow Batteries
The Vanadium Redox Battery (VRB®)¹ is a true redox flow battery (RFB), which stores energy by employing vanadium redox couples (V2+/V3+ in the negative and V4+/V5+ in the positive half-cells).
These active chemical species are fully dissolved at all times in sulfuric acid electrolyte solutions. Like other true RFBs, the power and energy ratings of Vanadium Redox Batteries are independent of each other and each may be optimized separately for a specific application. All the other benefits and distinctions of true RFBs compared to other energy storage systems are realized by VRBs. The first operational vanadium redox battery was successfully demonstrated at the University of New South Wales in the late 1980s and commercial versions have been operating on scale for over 8 years.
How Vanadium Flow Redox Batteries Work
During the discharge cycle, V2+ is oxidized to V3+ in the negative half-cell and an electron is released to do work in the external circuit (either DC or, for AC systems, through an AC/DC converter).
In the positive half-cell, V5+ in the form of VO2+ accepts an electron from the external circuit and is reduced to V4+ in the form of VO2+. Hydrogen (H+) ions are exchanged between the two half-cells to maintain charge neutrality. The hydrogen ions diffuse through the anion or cation-ion permeable polymer membrane that separates the half cells. Charged vanadium species and water can also diffuse across the membrane. The cross-diffusion results in direct energy loss for that cycle. However, when vanadium is the only element present on both sides of the cell, this cross diffusion mechanism does not result in permanent capacity loss, as long as the total vanadium in the system remains constant (i.e., there is no loss due to precipitation).
In a V-only system there is no need to maintain balance between positive and negative sides of the system. In the positive half-cell, the vanadium is present in solution as oxy-cations. These oxy-cations are vulnerable to irreversible precipitation as V2O5 if the electrolyte temperature exceeds ~50-60oC. However, when precipitation occurs, it does so typically in the form of benign compounds, not V2O5.
The normal operating temperature of a VRB is approximately between 10-40oC. Active cooling sub-systems are employed if ambient temperatures exceed 40-45oC. Being able to cool the system actively is an advantage since the system can remain operating without risking any damage to it. By contrast, if integrated cell architectures overheat, the best option is to stop using them until they cool down.
The cell voltage is 1.4-1.6 volts and cell power densities are hundreds mW/cm2 (although Prudent Energy reports their power densities are higher). The DC-DC efficiency of this battery has been reported in the range of 60-80%. According to EPRI, the vanadium redox battery is suitable for power systems in the range of 100 kW to 10 MW, with storage durations in the 2-8 hour range.
The vanadium redox battery offers a relatively high cell voltage, which is favorable for higher power and energy density compared with other true RFBs, like the iron-chromium system. However, the higher voltage and highly oxidative V5+ electrolyte puts more chemical stress on the materials used in the cell electrodes, membranes, and fluid handling components. Cross-transport of vanadium ions across the membrane is also reported as a challenge, and fairly expensive ion-exchange membranes must be used to minimize losses due to cross-membrane transport. These membranes can be vulnerable to fouling, wherein vanadium ions become irreversibly trapped in the membrane and increase resistive losses in the cell. On the other hand, lower cost membranes are under development.
Vanadium is a readily available material, used in steel manufacturing and as a chemical catalyst, which is found naturally and can also be recovered from various waste streams. The market price of vanadium as V2O5 has, however, been fairly volatile since 2017 after enjoying several years of low prices..
Notes
¹ VRB®, VRB-ESS®, and VRB ENERGY STORAGE SYSTEM® are registered trademarks of JD Holding, Inc., the parent company of Prudent Energy Corporation, a Delaware corporation. JD Holding, Inc. is the owner of U.S. Patent Nos. 6,143,443, 6,468,688, 6,562,514, 7,078,123, 7,181,183, 7,184,903, 7,227,275, 7,265,456, 7,353,083, 7,389,189, 7,517,608 and corresponding foreign patents. Additional patent rights are pending.
Nickel-Cadmium (NI-CD) batteries
In commercial production since the 1910s, nickel-cadmium (Ni-Cd) is a traditional battery type that has seen periodic advances in electrode technology and packaging in order to remain viable.
While not exceling in typical measures such as energy density or first cost, Ni-Cd batteries remain relevant by providing simple implementation without complex management systems, while providing long life and reliable service.
Sodium Sulfur (NaS) batteries
Sodium Sulfur (NaS) Batteries were originally developed by Ford Motor Company in the 1960s and subsequently the technology was sold to the Japanese company NGK.
NGK now manufactures the battery systems for stationary applications. The systems operate at a high temperature, 300 to 350 °C, which can be an operational issue for intermittent operation. Significant installations for energy storage have been used to facilitate distribution line construction deferral. The round trip efficiency is in the 90% range so provides an efficient use of energy.
Electrochemical Capacitors
Electrochemical capacitors (ECs) – sometimes referred to as “electric double-layer” capacitors – also appear under trade names like “Supercapacitor” or “Ultracapacitor.”
The phrase “double-layer” refers to their physically storing electrical charge at a surface-electrolyte interface of high-surface-area carbon electrodes. There are two types of ECs, symmetric and asymmetric, with different properties suitable for different applications. Markets and applications for electrochemical capacitors are growing rapidly and applications related to electricity grid will be part of that growth.
Iron-Chromium (ICB) Flow Batteries
Iron-chromium flow batteries were pioneered and studied extensively by NASA in the 1970s – 1980s and by Mitsui in Japan.
The iron-chromium flow battery is a redox flow battery (RFB). Energy is stored by employing the Fe2+ – Fe3+ and Cr2+ – Cr3+ redox couples. The active chemical species are fully dissolved in the aqueous electrolyte at all times. Like other true RFBs, the power and energy ratings of the iron-chromium system are independent of each other, and each may be optimized separately for each application. All the other benefits and distinctions of true RFBs compared to other energy storage systems are realized by iron-chromium RFBs.
Zinc-Bromine (ZNBR) Flow Batteries
The zinc-bromine battery is a hybrid redox flow battery, because much of the energy is stored by plating zinc metal as a solid onto the anode plates in the electrochemical stack during charge.
Thus, the total energy storage capacity of the system is dependent on both the stack size (electrode area) and the size of the electrolyte storage reservoirs. As such, the power and energy ratings of the zinc-bromine flow battery are not fully decoupled. The zinc-bromine flow battery was developed by Exxon as a hybrid flow battery system in the early 1970s.
Join American Clean Power
Elevate Your Business with Insider Access:
- Policy Direction: Your voice in critical industry discussions.
- Exclusive Networking: Learn directly from key players in clean energy.
- Insider Information: First-hand access to policy insights and premier events.
- Advanced Tools: Our proprietary data at your fingertips to drive growth.
Act now to become a defining part of clean energy’s future.
Stay informed
Subscribe to American Clean Power and receive the latest clean energy news, policy updates, and opportunities to get involved.